Presbyopia Is a Widespread, Undertreated, and Misunderstood Disease
Presbyopia is defined as the eye’s loss of ability to focus on objects at near distances, and it is the most common cause of age-related vision impairment. Presbyopia is a significant global health issue that is underdiagnosed. It affects all people in their 40s and older: an estimated 90 million people in the United States and more than 1 billion people worldwide, are affected.1 Of these, half a billion have no or inadequate spectacles, and 410 million are unable to perform important near tasks.1–3 Thus, presbyopia is both widespread and undertreated.
This disease is further complicated by lack of understanding of its progression and development. It is widely believed to be an unavoidable age-related condition instead of a preventable disease; as such, treatment options are mostly compensatory and not restorative. Such treatments often focus on refractive error, however accommodation is, at a fundamental level, a function involving biomechanics, movement, and shape changes. The movement and shape changes of the lens and other ocular structures allow a shift in focus to and from distant and near objects, as well as every distance in between. Historical presbyopic solutions are all compensatory treatments focusing only on the near refraction end goal, including reading spectacles. In addition, these corneal and intraocular lens approaches are static and fail to address the progressive loss of the dynamic movement that makes dynamic range of focus (DRoF) possible.
DRoF is the normal, prepresbyopic condition of the eye. The term includes the elements of the eye that provide a continuous range of focus instead of discreet near and far foci. DRoF involves neuromuscular and optical-based responses to stimuli. DRoF has optical, biomechanical, and neural components, which is why “visual perception” is quite unique from one individual to another.4 Age-related changes in the connective tissues, muscles, and even reflexivity to light stimuli all impact the eye’s ability and efficiency for DRoF function. These biomechanical dysfunctions also progress with age. Therefore, presbyopia results in a progressive loss of DRoF, a dysfunction that cannot be addressed with compensatory lenses or exchange of power at the cornea or lens to achieve near vision. These solutions only offer symptomatic relief, along with a compromise in other visual focal points.
Biomechanics and Ocular Rigidity Play an Important Role in Presbyopia Development
Although the cause of presbyopia is not fully understood, age-related changes to ocular connective tissue biomechanics play a major role.5,6 Traditional teaching is that presbyopia is due to stiffening of the lens and lens capsule7: as the lens and lens capsule become stiffer with age, the ciliary muscle is unable to produce sufficient resultant forces to overcome this added resistance to move and shape the lens into an accommodated position to achieve DRoF for near vision tasks. However, more recent studies demonstrate the importance of extralenticular tissues to DRoF and how they may contribute to presbyopia.5,6
Ocular rigidity (OR) is the globe’s ability to resist pressure in response to volume increases, and it also undergoes changes associated with age.8–13 Due to the change in scleral biomechanical properties,8,14 overall OR increases with age, and it is correlated with a decline in DRoF and is therefore a likely culprit causing the loss of DRoF function. The sclera, for example, exerts pressure on the underlying ciliary muscle and influences the overall rigidity of the eye.8,14 Scleral movement is also apparently important during accommodation. For example, the sclera near the limbus in the nasal region notches inward during accommodation in young healthy eyes, but this movement is lacking or diminished in presbyopes.15 This loss of movement is likely associated with the natural increasing scleral stiffness observed with age.16–20 It is possible that OR affects the pathogenesis and clinical course of various ocular conditions, including glaucoma, age-related macular degeneration (AMD), presbyopia, cataract formation, and corneal changes following refractive surgery.21
Remembering the importance of movement in accommodation, these tissues must maintain proper elasticity to achieve DRoF, but age is associated with loss of tissue elasticity. The Bruch’s membrane-choroid complex (BMCC) and retina also play important roles in DRoF and contribute to accommodative movements, including acting as an elastic spring to store energy and pull the lens backward during disaccommodation (near to far focus).22–24 These accommodation biodynamics are controlled by the neuromuscular function of the accommodation apparatus. These connective tissues are also affected by age in predictable ways, like all other tissues. The structures of the BMCC undergo increased stiffening with age,25 which is associated with a loss of elastic “spring” force and contributes to the loss of DRoF that occurs with presbyopia.24
This increasingly problematic biomechanical dysfunction induces mechanical impingement on the structures that play key roles in the eye’s ability to control intraocular pressure (IOP), such as the scleral spur, Schlemm’s canal, and the trabecular meshwork.26 These structures all work together to modulate IOP and regulate the hydrodynamic functions of the eye. The integration of these biomechanical components impact overall ocular health and fitness and is especially susceptible to age-related change and dysfunction.
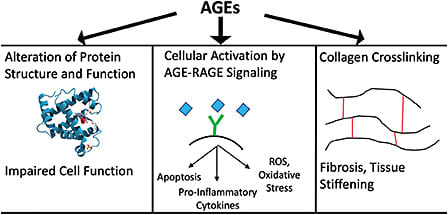
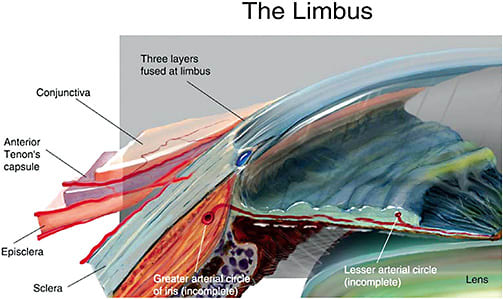
Collagen Crosslinking Is the Molecular Underpinning of Scleral and Ocular Rigidity
The biomechanical properties of ocular tissue, and therefore lens stiffness, capsular stiffness, and ocular rigidity, are largely determined by collagen. Collagen is a ubiquitous extracellular matrix protein of connective tissue, and its structure lends elasticity properties to the tissue with which it is associated.27 For example, the stress-strain curves of the sclera, which have shallow “toe” and steep “heel” sections, are formed as collagen fibers uncrimp and begin to bear their load uniformly across fibers.27,28
An important structural element of collagen fibers is crosslinking, in which adjacent fibers become covalently linked to each other via lysine residues. While some collagen crosslinking is normal in young, healthy eyes, collagen crosslinking increases with age.16,29,30 This process occurs in all ocular connective tissues as the production of advanced glycation end products (AGEs) increases with age. This increased AGE-induced crosslinking is a major contributor to lens and scleral stiffness, which in turn cause cataracts and ocular rigidity.16 AGEs have been identified as a trigger for oxidative stress and inflammation affecting organ health and function in other parts of the body, including the heart and brain.31
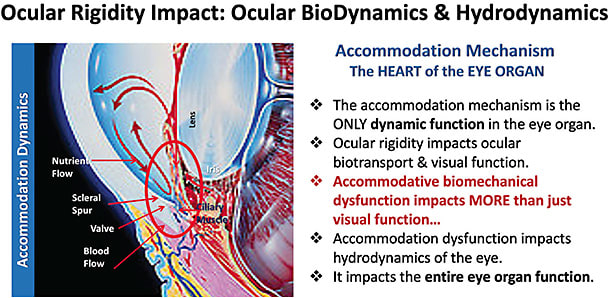
Could Ocular Rigidity Underlie Multiple Diseases of the Aging Eye?
Presbyopia is not the only age-related eye disease: cataracts, AMD, primary open angle glaucoma, and diabetic retinopathy occur with increasing frequency with age and are correlated with the onset and progressive problem of AGEs.32–34 Moreover, AGEs have been established as biomarkers for all ocular pathologies, including progressive lens stiffness and progressive ocular rigidity in presbyopia, as well as lens death, which produces cataracts.33,34 Presbyopia has been proposed to be an early symptom of cataract.35 Multiple diseases have been associated with increasing ocular rigidity, including presbyopia, glaucoma, and AMD.21
Moreover, the movements associated with DRoF might be critical to maintaining other parts of the eye in a healthy state. The crystalline lens, along with extralenticular structures of the accommodative apparatus, move in a fluid medium (aqueous humor) and via that medium cause transient pressure changes and hydrodynamic movements during accommodation. These neuromuscular and biomechanical structures within the accommodative complex also impose stretch forces upon important structures, such as the trabecular meshwork, during ciliary muscle contraction, during accommodation and disaccommodation.
Finally, accommodative movements that produce DRoF are also physiologically important for maintaining appropriate aqueous outflow, IOP hydrodynamics, and nutrient bathing of the lens in young, healthy eyes through the ciliaris, scleral spur, and trabecular meshwork. Therefore, the loss of efficient DRoF function is a gateway to multiple ocular pathologies when age-related biomechanical dysfunction occurs.36 This underlines the critical importance of restoring DRoF, which potentially provides an ocular health benefit, in addition to functional compensation, rather than simply providing refractory compensation. For this reason, attempting to restore DRoF might also have an added ocular health benefit, in addition to dynamic focusing ability. In consideration of the various physiological interactions in which the structures of the accommodation complex participate, restoring DRoF has the potential to delay the onset of other ocular diseases of advanced age. Restoring DRoF has clinical significance; therefore, it should be pursued. ■
References
- Fricke TR, Tahhan N, Resnikoff S, et al. Global prevalence of presbyopia and vision impairment from uncorrected presbyopia: systematic review, meta-analysis, and modelling. Ophthalmology. 2018;125(10):1492-1499.
- Holden BA, Fricke TR, Ho SM, et al. Global vision impairment due to uncorrected presbyopia. Arch Ophthalmol. 2008;126(12):1731-1739.
- Bourne RRA, Flaxman SR, Braithwaite T, et al. Magnitude, temporal trends, and projections of the global prevalence of blindness and distance and near vision impairment: a systematic review and meta-analysis. Lancet Glob Health. 2017;5(9):e888-e897.
- Plainis S, Charman WN, Pallikaris IG. The physiologic mechanism of accommodation. CRSTEurope website. April 2014. Accessed November 16, 2022. https://crstodayeurope.com/articles/2014-apr/the-physiologic-mechanism-of-accommodation/
- Tamm E, Croft MA, Jungkunz W, Lütjen-Drecoll E, Kaufman PL. Age-related loss of ciliary muscle mobility in the rhesus monkey. Role of the choroid. Arch Ophthalmol. 1992;110(6):871-876.
- Croft MA, Glasser A, Kaufman PL. Accommodation and presbyopia. Int Ophthalmol Clin. 2001;41(2):33-46.
- Croft MA, Kaufman PL. Accommodation and presbyopia: the ciliary neuromuscular view. Ophthalmol Clin North Am. 2006;19(1):13-24, v.
- Kotliar K. Ocular rigidity: clinical approach. In: Pallikaris I, Tsilimbaris MK, Dastiridou AI, eds. Ocular Rigidity, Biomechanics and Hydrodynamics of the Eye. Springer International Publishing; 2021:15-43.
- Friedenwald JS. Contribution to the Theory and Practice of Tonometry. Am J Ophthalmol. 1937;20(10):985-1024.
- Kontadakis G, Kymionis G. Age related changes in ocular rigidity. In: Pallikaris I, Tsilimbaris MK, Dastiridou AI, eds. Ocular Rigidity, Biomechanics and Hydrodynamics of the Eye. Springer International Publishing; 2021:221-226.
- Perkins ES. Ocular volume and ocular rigidity. Exp Eye Res. 1981;33(2):141-145.
- Gaasterland D, Kupfer C, Milton R, Ross K, McCain L, MacLellan H. Studies of aqueous humour dynamics in man. VI. Effect of age upon parameters of intraocular pressure in normal human eyes. Exp Eye Res. 1978;26(6):651-656.
- Pallikaris IG, Kymionis GD, Ginis HS, Kounis GA, Tsilimbaris MK. Ocular rigidity in living human eyes. Invest Ophthalmol Vis Sci. 2005;46(2):409-414.
- Hommer A, Fuchsjäger-Mayrl G, Resch H, Vass C, Garhofer G, Schmetterer L. Estimation of ocular rigidity based on measurement of pulse amplitude using pneumotonometry and fundus pulse using laser interferometry in glaucoma. Invest Ophthalmol Vis Sci. 2008;49(9):4046-4050.
- Croft MA, Nork TM, McDonald JP, Katz A, Lütjen-Drecoll E, Kaufman PL. Accommodative movements of the vitreous membrane, choroid, and sclera in young and presbyopic human and nonhuman primate eyes. Invest Ophthalmol Vis Sci. 2013;54(7):5049-5058.
- Watson PG, Young RD. Scleral structure, organisation and disease. A review. Exp Eye Res. 2004;78(3):609-623.
- Friberg TR, Lace JW. A comparison of the elastic properties of human choroid and sclera. Exp Eye Res. 1988;47(3):429-436.
- Avetisov ES, Savitskaya NF, Vinetskaya MI, Iomdina EN. A study of biochemical and biomechanical qualities of normal and myopic eye sclera in humans of different age groups. Metab Pediatr Syst Ophthalmol. 1983;7(4):183-188.
- Coudrillier B, Tian J, Alexander S, Myers KM, Quigley HA, Nguyen TD. Biomechanics of the human posterior sclera: age- and glaucoma-related changes measured using inflation testing. Invest Ophthalmol Vis Sci. 2012;53(4):1714-1728.
- Geraghty B, Jones SW, Rama P, Akhtar R, Elsheikh A. Age-related variations in the biomechanical properties of human sclera. J Mech Behav Biomed Mater. 2012;16:181-191.
- Detorakis ET, Pallikaris IG. Ocular rigidity: biomechanical role, in vivo measurements and clinical significance. Clin Exp Ophthalmol. 2013;41(1):73-81.
- Goldberg DB. Biomechanics of the lens and hydrodynamics of accommodation. In: Pallikaris I, Tsilimbaris MK, Dastiridou AI, eds. Ocular Rigidity, Biomechanics and Hydrodynamics of the Eye. Springer International Publishing; 2021:117-126.
- Bassnett S. Zinn’s zonule. Prog Retin Eye Res. 2021;82:100902.
- Strenk LM, Guo S, Lu K, Werner L, Strenk SA. Force of lifelong crystalline lens growth: chronic traumatic mechanical insult to the choroid. J Cataract Refract Surg. 2022;48(3):342-348.
- Ugarte M, Hussain AA, Marshall J. An experimental study of the elastic properties of the human Bruch’s membrane-choroid complex: relevance to ageing. Br J Ophthalmol. 2006;90(5):621-626.
- Boote C, Sigal IA, Grytz R, Hua Y, Nguyen TD, Girard MJA. Scleral structure and biomechanics. Prog Retin Eye Res. 2020;74:100773.
- Grytz R, Fazio MA, Libertiaux V, et al. Age- and race-related differences in human scleral material properties. Invest Ophthalmol Vis Sci. 2014;55(12):8163-8172.
- Boazak EM, Ethier CR. Basic Engineering concepts and terminology underlying ocular rigidity. In: Pallikaris I, Tsilimbaris MK, Dastiridou AI, eds. Ocular Rigidity, Biomechanics and Hydrodynamics of the Eye. Springer International Publishing; 2021:1-13.
- Ihanamäki T, Salminen H, Säämänen AM, et al. Age-dependent changes in the expression of matrix components in the mouse eye. Exp Eye Res. 2001;72(4):423-431.
- Campbell IC, Lovald S, Garcia M, Coudrillier B. Biomechanical properties of the sclera. In: Pallikaris I, Tsilimbaris MK, Dastiridou AI, eds. Ocular Rigidity, Biomechanics and Hydrodynamics of the Eye. Springer International Publishing; 2021:77-105.
- Venkataraman K, Khurana S, Tai TC. Oxidative stress in aging--matters of the heart and mind. Int J Mol Sci. 2013;14(9):17897-17925.
- Nandi SK, Nahomi RB, Rankenberg J, Glomb MA, Nagaraj RH. Glycation-mediated inter-protein cross-linking is promoted by chaperone-client complexes of α-crystallin: Implications for lens aging and presbyopia. J Biol Chem. 2020;295(17):5701-5716.
- Stitt AW. Advanced glycation: an important pathological event in diabetic and age related ocular disease. Br J Ophthalmol. 2001;85(6):746-753.
- Kandarakis SA, Piperi C, Topouzis F, Papavassiliou AG. Emerging role of advanced glycation-end products (AGEs) in the pathobiology of eye diseases. Prog Retin Eye Res. 2014;42:85-102.
- McGinty SJ, Truscott RJW. Presbyopia: the first stage of nuclear cataract? Ophthalmic Res. 2006;38(3):137-148.
- Goel M, Picciani RG, Lee RK, Bhattacharya SK. Aqueous humor dynamics: a review. Open Ophthalmol J. 2010;4:52-59.