Introduction
PRESBYOPIA AFFECTS nearly everyone older than 50, resulting in near vision impairment for more than a billion people.1–3 Many of these cases are untreated, and the economic burden of presbyopia is high, as it is the most common cause of visual impairment worldwide.4 The development of presbyopia therapies must therefore be a major priority for ophthalmologic research. For sustained progress in such therapeutic research, however, we must have a proper understanding of the underlying causes of presbyopia.
In the first two installments of this series, we outlined the biomechanics of Dynamic Range of Focus (DRoF), the eye’s ability to adjust focus across variable distances.5,6 This biomechanical description helps us to understand how the eye can change focus from Phase 0 (fully disaccommodated) to Phase 1 (moving from disaccommodated to accommodated) to Phase 2 (moving from accommodated to disaccommodated). It explicitly links the focusing function of the eye with tissue biomechanics through a kinematic chain. Until now, we have focused on the functioning of this kinematic chain in healthy eyes, but we have not explicitly examined the effects of aging on the biomechanics of this system. Here, we conclude this series by showing how the aging biomechanics of the eye lead to the disease we call presbyopia.
Aging and Crosslinking in Connective Tissues
The major structural protein of connective tissues is collagen. Collagen is a triple helical protein that is further organized into fibrils made of multiple collagen molecules in a typical repeating pattern.7,8 These fibrils are held together with crosslinks, whereby nearby lysine residues become covalently bound. Crosslinks also occur within the helix and affect the flexibility of the protein through conversion of sulfhydryl groups (which are flexible) into disulfide bonds (which are rigid). This crosslinking is a normal feature of collagen, and it reduces elasticity to provide more structure. However, aging is associated with disruption of the normal amount of crosslinking, leading to the increased stiffness observed across tissues during aging.9–11 Reducing sugars, such as glucose, interact with collagen to cause glycation events, and through subsequent reactions, they produce long-lived advanced glycation end products (AGEs), which introduce crosslinks into the helical portion of collagen.12,13 As these crosslinks build up with aging, tissue stiffness increases.12,14 Tissue damage, reduced matrix production, and reduced collagen turnover may all play a role in this age-associated crosslinking.
Biomechanical Stiffness, Crosslinking, Ocular Rigidity, and Loss of DRoF
Many of the critical components of the biomechanical apparatus that performs the DRoF function are susceptible to the same aging processes that affect connective tissues in the rest of the body, notably tissue stiffening. Ocular rigidity as a whole increases with age;15 rigidity of the individual anatomical components associated with accommodation also increase, including the lens and lens capsule,16–19 sclera,20–24 and Bruch’s membrane-choroid complex (BMCC).25 These changes have a profound impact on the movements of these tissues and ultimately lead to presbyopia. Here we examine how this biomechanical stiffness affects each stage of DRoF.
Phases of DRoF: Presbyopic Eye
Prestretch: In the presbyopic eye, stiffness of the BMCC and lens prevents the lens from attaining its former range of motion. The lens is relatively rounder and forward-positioned, compared to a younger eye (Figures 1-3).
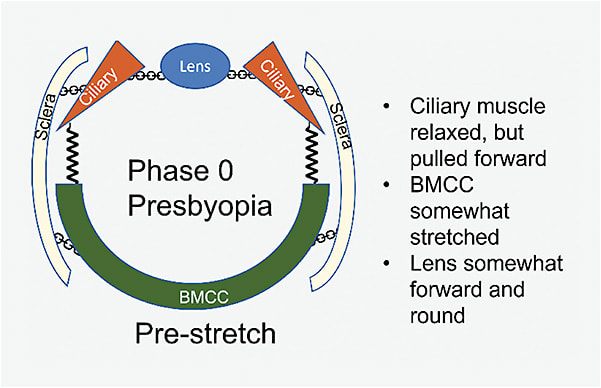
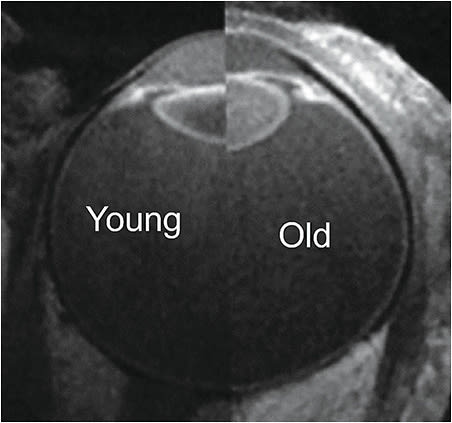
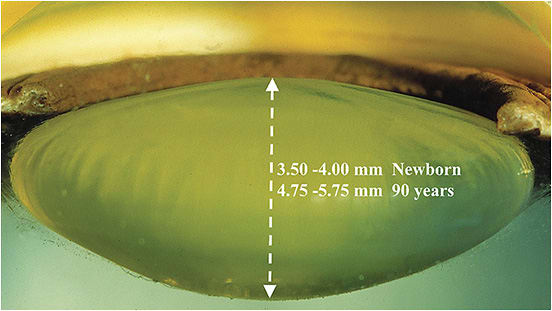
Accommodation: Although the ciliary muscle has sufficient force, tissue stiffness limits the movements of the accommodative apparatus (Figure 4). The BMCC is stiffer and pulls harder against the muscle in the backward direction. The sclera is stiffer and shows less inward bowing. Thus, despite the force generated by the ciliary muscle being sufficient to effect lens shape change in a young person, the stiff sclera and BMCC provide strong resistance to the ciliary muscle in the presbyopic eye. The lens shape changes less with aging, ultimately leading to lower optical power than what can be achieved in the young eye.
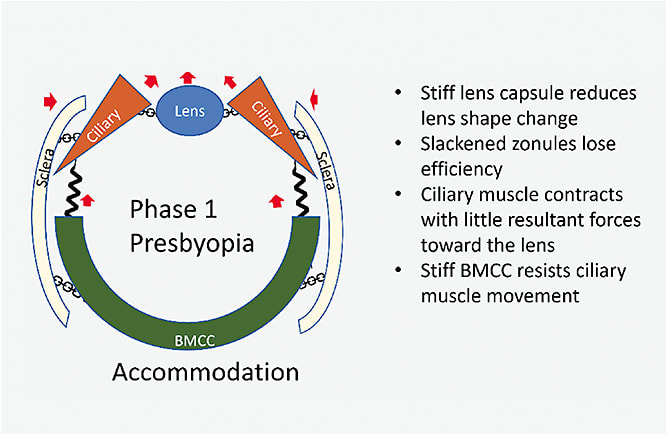
Disaccommodation: Increased stiffness of the BMCC prevents strong movement backward (Figure 5). Instead of acting like a recoiling spring, the stiffer BMCC dampens movement of the accommodative apparatus. Although relaxed, the ciliary muscle is unable to reshape the lens into full disaccommodation or achieve the full resting posture of a young eye. Thus, the lens is not pulled flat and stays in a relatively rounded position.
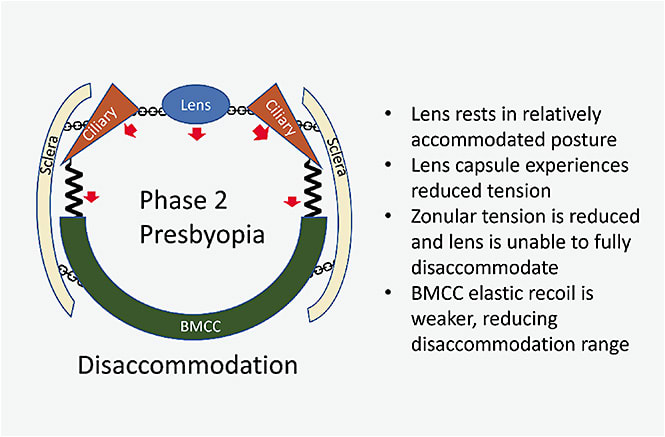
Conclusions
Accommodation involves a complex Kinematic Chain of anatomical components and movements. Aging causes increased stiffness in those components, ultimately leading to reduced shape-changing ability of the lens and a reduction in the optical power that can be achieved through all of the components of DRoF, including accommodation, pseudoaccommodation, extended depth of focus, and pupillary diaphragmatic changes. The DRoF biomechanical dysfunction that occurs with presbyopia affects more than just visual quality; it also affects the physiological functions of the eye.27–29 The development of therapies that address both the visual quality and reduced ocular functions associated with presbyopia will depend on recognizing the biomechanical effects of aging and finding ways to reverse the stiffness caused by aging. Addressing the pathogenesis of the aging eye, as we discussed in the original article of this series, will be required to understand the root cause of the problem of presbyopia and lead to solutions that actually treat the real problem of presbyopia and not only the symptoms.
Finally, presbyopia is an aging problem. Therefore, it is a progressive pathophysiological disease. Attempting to treat the progressive problem of presbyopia with a “one and done” solution, such as vision-correction remedies, has not worked to date and will likely not provide viable strategies for this aging disease. The Kinematic Chain of biomechanical events gives a framework for understanding both functional and dysfunctional events of DRoF that can illuminate the anatomical structures contributing to DRoF and the impact of age on these structures and the eye’s intricate functions. Solutions are needed that address the biomechanical dysfunctions that occur with consideration of the stiffening effects of AGEs impacting all ocular connective tissue, the sclera, the lens, and the extralenticular structures of the eye. ■
References
- Yang X, Chen H, Zhang T, et al. Global, regional, and national burden of blindness and vision loss due to common eye diseases along with its attributable risk factors from 1990 to 2019: a systematic analysis from the global burden of disease study 2019. Aging. 2021;13(15):19614-19642.
- Holden BA, Fricke TR, Ho SM, et al. Global vision impairment due to uncorrected presbyopia. Arch Ophthalmol. 2008;126(12):1731-1739.
- Fricke TR, Tahhan N, Resnikoff S, et al. Global prevalence of presbyopia and vision impairment from uncorrected presbyopia: systematic review, meta-analysis, and modelling. Ophthalmology. 2018;125(10):1492-1499.
- Berdahl J, Bala C, Dhariwal M, Lemp-Hull J, Thakker D, Jawla S. Patient and economic burden of presbyopia: a systematic literature review. Clin Ophthalmol. 2020;14:3439-3450.
- Hipsley A, Bocskai Z, Price E. Dynamic range of focus of the eye: understanding biomechanics and kinematics: part I. Presbyopia Physician. 2023;3(1):25-28, 43.
- Hipsley AM, Price ER, Bocskai ZI, Marshall J. Dynamic range of focus of the eye: understanding biomechanics and kinematics: part II. Presbyopia Physician. 2023;3(2):28-32.
- Shoulders MD, Raines RT. Collagen structure and stability. Annu Rev Biochem. 2009;78:929-958.
- Chang SW, Buehler MJ. Molecular biomechanics of collagen molecules. Mater Today. 2014;17(2):70-76.
- Watson PG, Young RD. Scleral structure, organisation and disease. A review. Exp Eye Res. 2004;78(3):609-623.
- Ihanamäki T, Salminen H, Säämänen AM, et al. Age-dependent changes in the expression of matrix components in the mouse eye. Exp Eye Res. 2001;72(4):423-431.
- Campbell IC, Lovald S, Garcia M, Coudrillier B. Biomechanical properties of the sclera. In: Pallikaris I, Tsilimbaris MK, Dastiridou AI, eds. Ocular Rigidity, Biomechanics and Hydrodynamics of the Eye. Springer International Publishing; 2021:77-105.
- Svensson RB, Smith ST, Moyer PJ, Magnusson SP. Effects of maturation and advanced glycation on tensile mechanics of collagen fibrils from rat tail and Achilles tendons. Acta Biomater. 2018;70:270-280.
- Vasan S, Zhang X, Zhang X, et al. An agent cleaving glucose-derived protein crosslinks in vitro and in vivo. Nature. 1996;382(6588):275-278.
- Bailey AJ, Paul RG, Knott L. Mechanisms of maturation and ageing of collagen. Mech Ageing Dev. 1998;106(1-2):1-56.
- Pallikaris IG, Kymionis GD, Ginis HS, Kounis GA, Tsilimbaris MK. Ocular rigidity in living human eyes. Invest Ophthalmol Vis Sci. 2005;46(2):409-414.
- Zhou H, Yan H, Yan W, Wang X, Li Q. In vivo ultrasound elastographic evaluation of the age-related change of human lens nuclear stiffness. BMC Ophthalmol. 2020;20(1):135.
- Krag S, Andreassen TT. Mechanical properties of the human posterior lens capsule. Invest Ophthalmol Vis Sci. 2003;44(2):691-696.
- Krag S, Olsen T, Andreassen TT. Biomechanical characteristics of the human anterior lens capsule in relation to age. Invest Ophthalmol Vis Sci. 1997;38(2):357-363.
- Avetisov KS, Bakhchieva NA, Avetisov SE, et al. Biomechanical properties of the lens capsule: a review. J Mech Behav Biomed Mater. 2020;103:103600.
- Friberg TR, Lace JW. A comparison of the elastic properties of human choroid and sclera. Exp Eye Res. 1988;47(3):429-436.
- Avetisov ES, Savitskaya NF, Vinetskaya MI, Iomdina EN. A study of biochemical and biomechanical qualities of normal and myopic eye sclera in humans of different age groups. Metab Pediatr Syst Ophthalmol. 1983;7(4):183-188.
- Coudrillier B, Tian J, Alexander S, Myers KM, Quigley HA, Nguyen TD. Biomechanics of the human posterior sclera: age- and glaucoma-related changes measured using inflation testing. Invest Ophthalmol Vis Sci. 2012;53(4):1714-1728.
- Geraghty B, Jones SW, Rama P, Akhtar R, Elsheikh A. Age-related variations in the biomechanical properties of human sclera. J Mech Behav Biomed Mater. 2012;16:181-191.
- Fazio MA, Grytz R, Morris JS, Bruno L, Girkin CA, Downs JC. Human scleral structural stiffness increases more rapidly with age in donors of African descent compared to donors of European descent. Invest Ophthalmol Vis Sci. 2014;55(11):7189-7198.
- Ugarte M, Hussain AA, Marshall J. An experimental study of the elastic properties of the human Bruch’s membrane-choroid complex: relevance to ageing. Br J Ophthalmol. 2006;90(5):621-626.
- Strenk LM, Guo S, Lu K, Werner L, Strenk SA. Force of lifelong crystalline lens growth: chronic traumatic mechanical insult to the choroid. J Cataract Refract Surg. 2022;48(3):342-348.
- Last JA, Pan T, Ding Y, et al. Elastic modulus determination of normal and glaucomatous human trabecular meshwork. Invest Ophthalmol Vis Sci. 2011;52(5):2147-2152.
- Sigal IA, Roberts MD, Girard M, Burgoyne CF, Downs JC. Biomechanical changes of the optic disc. In: Levin LA, Albert DM, eds. Ocular Disease: Mechanisms and Management. Elsevier; 2010:153-164.
- Tsilimbaris MK. Ocular rigidity and age related macular degeneration. In: Pallikaris I, Tsilimbaris MK, Dastiridou AI, eds. Ocular Rigidity, Biomechanics and Hydrodynamics of the Eye. Springer International Publishing; 2021:291-295.